Think Small: How quantum will change the world
CIFAR researchers in the Quantum Materials and Quantum Information Science programs are doing big things with tiny particles
We are on the cusp of another technological revolution.
To the ancient Greeks, the word ‘atomos’ means uncuttable, and the philosopher Democritus applied this idea to the smallest possible unit of matter. If you cut a material into ever-smaller pieces, until you can cut no more, then you have an atom.
The modern idea of the atom borrows this word, but we now know that there are particles far smaller than the atom itself. These subatomic particles defy explanation by classical physics, and new theory was needed to explain their bizarre properties. It’s called the theory of quantum mechanics, and in the early 20th century, it began to transform how we understood the workings of the universe, on the tiniest scale.
It has already enabled powerful technologies like computer chips and MRI machines. And now, quantum mechanics is being applied to the next generation of transformational tech.
Researchers in CIFAR’s Quantum Materials and Quantum Information Science programs are advancing quantum technologies that will revolutionize computing, electrical grids, transportation networks, and more.
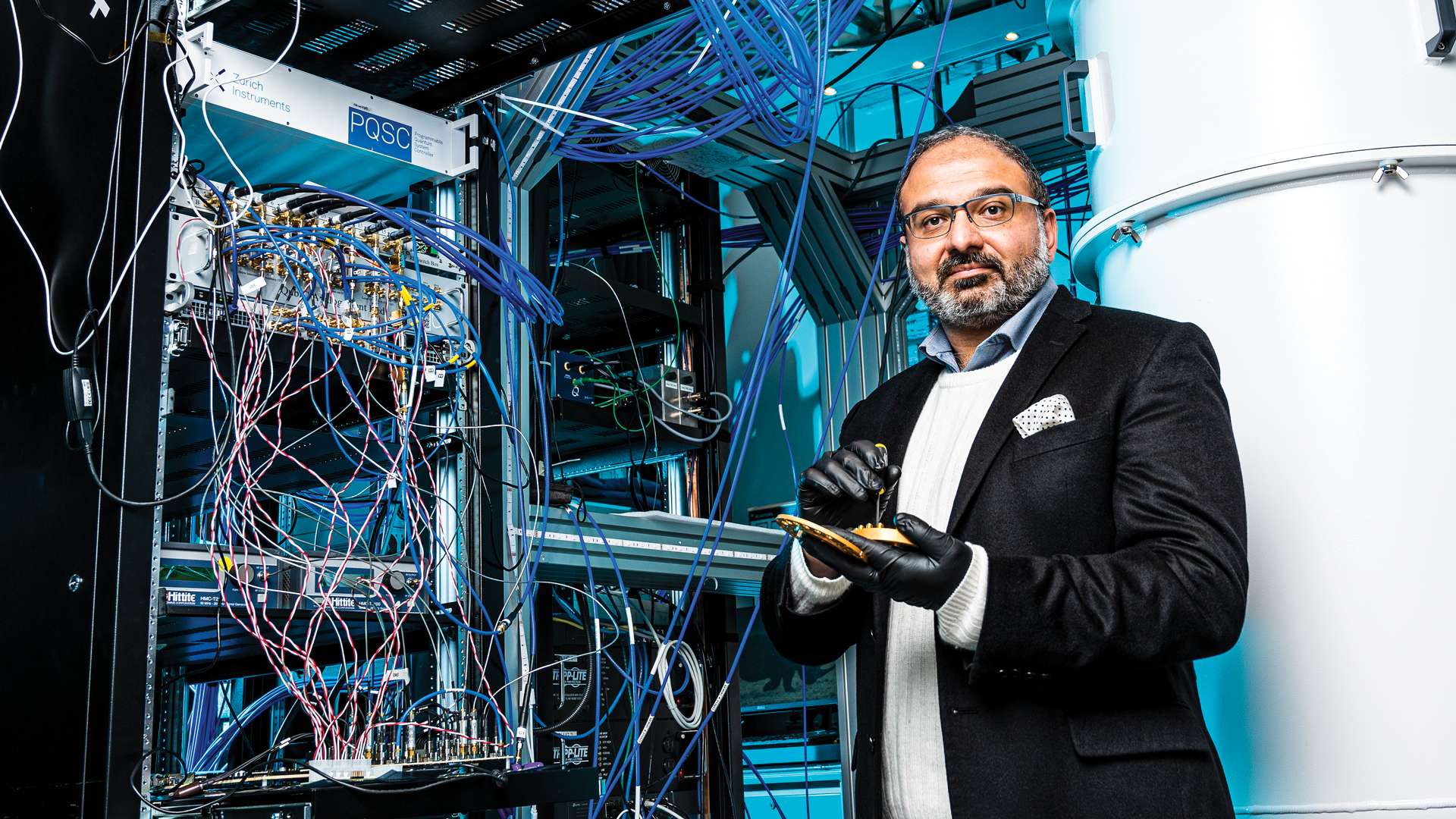
IRFAN SIDDIQI
When an electron leaps between orbits around an atom’s nucleus, a tiny particle of light is emitted. It is so faint that it’s barely perceptible, but this particle, called a photon, is predicted by quantum mechanics. It’s called a “quantum jump,” and when it occurs, the photon and atom can be linked by a phenomenon called quantum entanglement. Their physical properties are interdependent, even if the particles are far apart.
The concept is, well, kind of spooky. That’s how Albert Einstein described it. He argued that quantum entanglement was impossible because it allowed for the interdependence of particles, even if the distance between them is so great that light itself could not travel quickly enough to bridge it. Einstein called it “spooky action.” It seems to defy his theory of special relativity, which posits that nothing travels faster than light.
But the signatures of quantum entanglement can be observed, and it can even be created on demand. And it is central to unlocking the potential of quantum computing. Classical computing devices like laptops conduct operations using bits — made with transistors on a computer chip. Each bit can have a value of zero or one. It can be in either of these, but not both.
But a quantum computer makes computations with qubits — short for quantum bits. There are different approaches to physically creating these, but all use quantum mechanical phenomena to allow the qubit to be in a state of superposition. That means that it can be both a zero and a one at the same time.
And when multiple qubits are linked together through quantum entanglement, they can be operated on simultaneously. This increases computational power exponentially, and allows certain types of operations to be performed much more quickly.
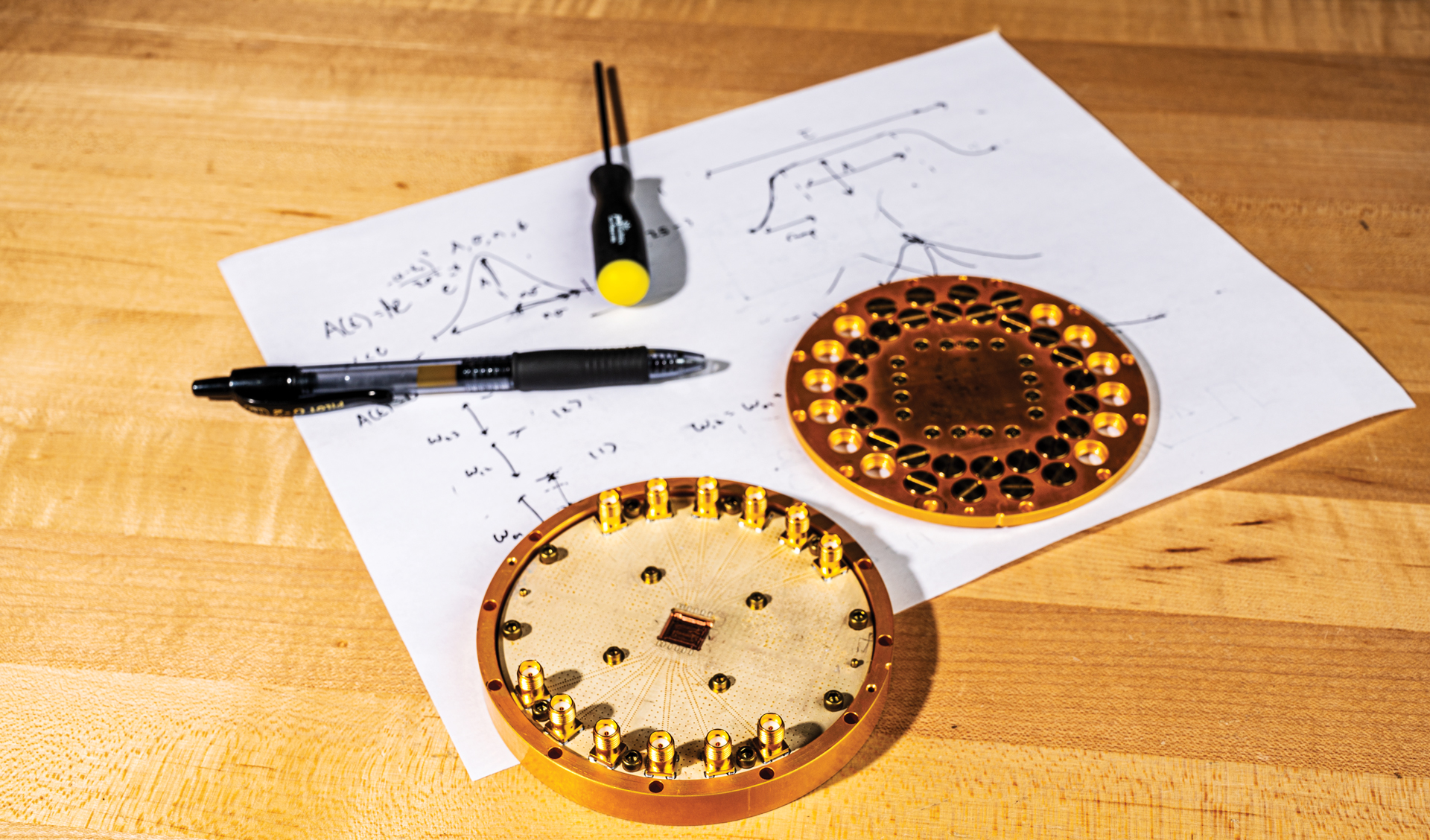
“In a classical computing device like a laptop, each transistor is independent — none interferes with the others,” says Irfan Siddiqi, a fellow in the Quantum Information Science program and a professor of Physics at the University of California Berkeley in the U.S.
“But qubits are entangled with each other. This could allow them to process information more effectively. It is the promise of quantum mechanics, but we need to create entanglement on a large scale. We can do a hundred qubits, but we need to entangle a hundred thousand, even a million. And we don’t know how to create and control qubits at that scale.”
Creating entanglement requires a highly controlled setting. Quantum computers are especially vulnerable to interference that researchers call “noise,” which causes errors and disrupts quantum entanglement. Error-causing “noise” can be anything from light to radiation from nearby wi-fi networks.
“Quantum information lasts a very short time, but there are ways to extend its lifetime. You can make the materials and everything else perfect, so that there are no errors and no noise. We have been trying that for more than 20 years, and it’s pretty hard. You need to get all of the pieces right. Otherwise, it doesn’t work,” says Siddiqi.
Siddiqi uses magnetism to control quantum information, and this can help reduce errors. It helps prevent certain types of noise because magnetism couples more weakly than electric charges.
“We should design quantum infor-mation systems so only certain types of errors occur, then pair them with practical error correction methods,” says Siddiqi.
“This makes error correction easier. You might only have to correct for one type of error. Whereas correcting for arbitrary types of error is hard. There are many ways something can go wrong. All it takes is a photon or even a cosmic ray.”
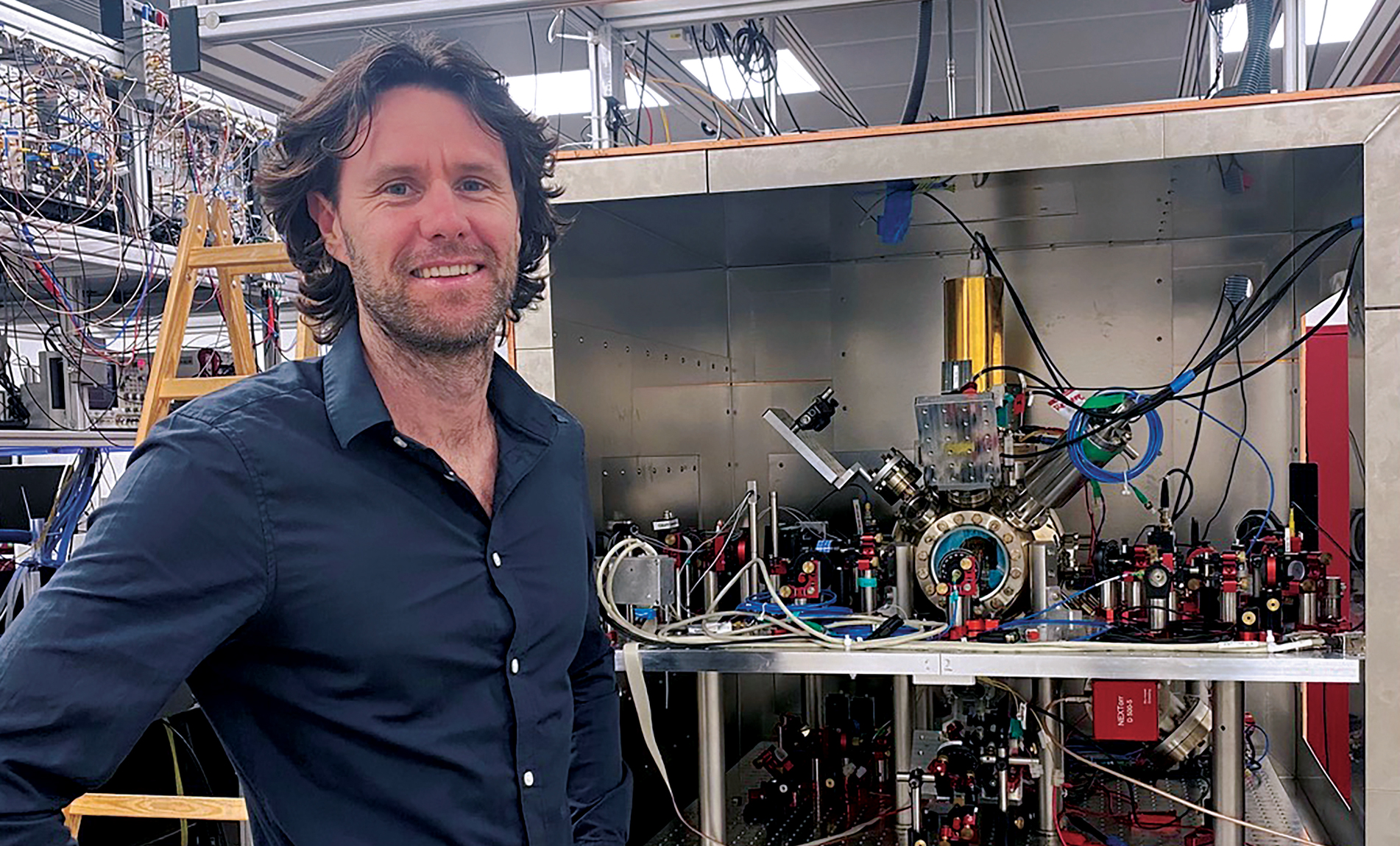
BEN LANYON
The trapped ion chamber of Ben Lanyon’s quantum computer is made of titanium, plated in gold, and bonded by sapphire. It uses electric fields to suspend electrically charged calcium atoms called ions, and manipulates their quantum state with lasers.
Trapped ion systems are among the most promising approaches to quantum computing.
“One reason is their level of proven quantum control,” says Lanyon, a fellow in the Quantum Information Science program and an assistant professor at the University of Innsbruck in Austria.
“We can precisely manipulate ions, and get them to evolve into very interesting quantum states that we do not properly understand yet. But you do not get anything for free, and trapped ion quantum computers are not as easily scalable as some approaches to quantum computing could be.”
Researchers have scaled this system to trap tens of ions, and harness their quantum states to use as qubits. But there is no path to directly scale up this type of system to the thousands or millions of qubits needed to perform some applications.
So instead of making trapped ion quantum computers larger, Lanyon is linking them together.
“We can precisely manipulate ions, and get them to evolve into very
interesting quantum states that we do not properly understand yet.”
— Ben Lanyon
This is a step toward distributed quantum networks, which have near-term applications in computation, communications security and atomic clocks.
The building blocks of quantum networks are already emerging, and, in collaboration with colleague Tracy Northup at University of Innsbruck, Lanyon is using light to create links between ions that are hundreds of metres apart.
A recent experiment linked two University of Innsbruck ion trap quantum computers with fibre optic cable — the same commercial glass cable used in existing communications networks. And inside each computer’s 2-centimetre-long cavity, a single calcium ion was suspended in an electric field. These were surrounded by small mirrors, and lasers induced each ion to emit a photon — a single particle of light.
The photons bounced around inside each chamber tens of thousands of times before emerging through a mirror and into the cable. One mirror was slightly weaker than the other, and that mirror connected to the cable. Once the photons passed through their respective mirrors, they hurtled toward each other at the speed of light, meeting in the middle. That’s where quantum theory kicks in.
“When the calcium ion produces the photon, the two are entangled. And when it leaks out the side of the chamber, they remain entangled. When the two photons met in the cable, they were still entangled with the ions that produced them,” says Lanyon.
“We can detect these particles with a photon detector — and if you do that just right, it will destroy them. And when these photons are destroyed together, the entanglement gets swapped from the ion-photon pairs to the two remote ions.”
Though the remote ions in the trapped ion chambers were hundreds of metres apart, they maintained an entangled state — a step toward building quantum networks that link together quantum computers that stretch across cities, countries and even continents.
“Entangling atoms over a distance of 100 kilometres would be meaningful. That is only six steps to Vienna, or a further 10 to Paris,” says Lanyon.
“The real question is how to practically and reliably do this. Then we could distribute and store entanglement across Europe — a new resource for science and technology.”
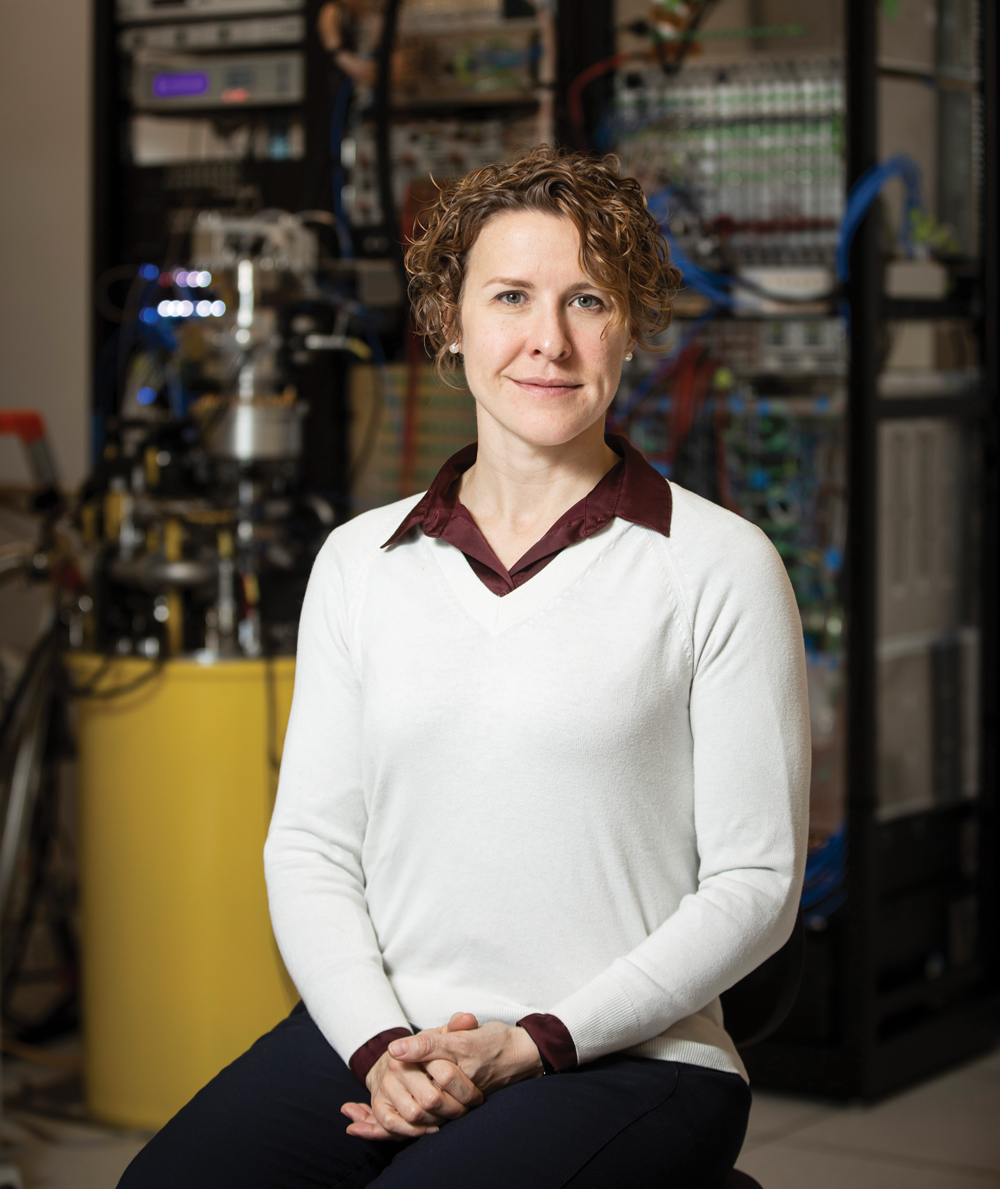
STEPHANIE SIMMONS
Silicon has been the backbone of the information revolution. Many billion silicon computer chips are manufactured each year, and they power everything from mobile phones to laptop computers to smart refrigerators. Silicon chips are everywhere — a single automobile can have as many as 3,000 of them.
But Stephanie Simmons, a fellow in the Quantum Information Science program, believes that silicon computer chips can do much more than they are today. She is using silicon to power quantum computing.
Silicon’s advantage is its ubiquity. We already know how to work with it, and Simmons is using its known properties to create qubits. To do this, Simmons uses natural defects in silicon called colour centres. Using these defects, it is possible to make computations with a type of qubit called a spin qubit. Its spin is controlled with magnetism, and the spin qubits can absorb or emit photons, which allows them to communicate with each other. This is not possible with traditional computing chips, on which each bit functions independently.
“New technologies go from being magic to boring, and that’s the vision for quantum. We want to make it routine.” — Stephanie Simmons
“I thought we would be looking for the right kind of colour centres for decades,” says Simmons, a Professor of Physics at Simon Fraser University (SFU) in Canada.
“It had to be something that formed naturally. You take what nature gives you, because otherwise it will be difficult to produce qubits with enough uniformity, quality and scale. I won’t necessarily be able to engineer an entirely new defect, but many form naturally. And when you use these as the quantum backbone, you can basically print them at scale, right into silicon chips. There has been decades of development in silicon, and that matters for scalability.”
Quantum computing was first proposed by the American physicist Richard Feynman in the 1980s. Quantum mechanics is too complicated to be computed on classical computers because of the large number of dynamic interactions at the atomic and subatomic level. Even with today’s powerful computing technology, researchers use approximations to conduct these calculations.
Feynman argued that a quantum mechanical machine might overcome this, but building one hasn’t been easy. No dominant design has emerged, but Simmons believes silicon has what it takes.In2016, she co-founded Photonic Inc. with SFU Professor Emeritus Mike Thewalt, and the Vancouver-based company is developing quantum computing and networking technology to deploy at scale.
“We think of bits as abstract, but they are actually physically in silicon, moving and changing state. Ultimately, information is a physical thing,” says Simmons.
“There has been a worldwide race to harness and commercialize quantum physics. And historically, every time we have seen something like this, it has been transformational and unpredictable. Two centuries ago, people filled auditoriums to see magnets. Now, we don’t think twice about electromagnetism, even though we use it every time we plug in our devices. New technologies go from being magic to boring, and that’s the vision for quantum. We want to make it routine.”
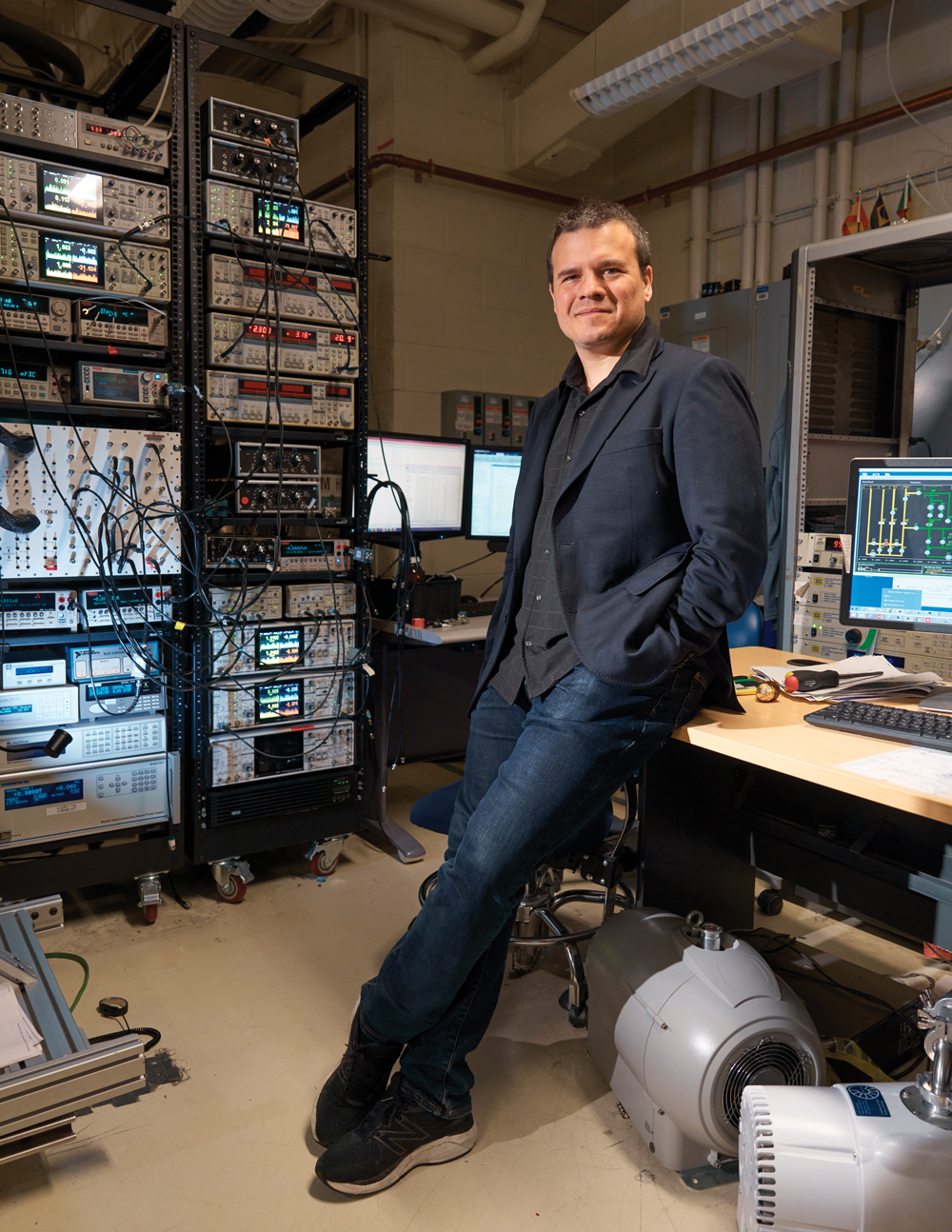
PABLO JARILLO-HERRERO
Graphene is the strongest material known to exist. It is a layer of graphite just a single atom thick — so thin it’s called a two-dimensional material. Graphene is also extremely flexible, and already used in electronics like bend-able mobile phones.
One day, graphene might allow you to wrap a tablet around your wrist like a smart watch — then easily return it to its original shape. But graphene could have even more intriguing applications than that, and Pablo Jarillo-Herrero is probing the material’s quantum properties to better understand what those could be.
“Graphene comes from graphite, which is three-dimensional. But it is like a deck of cards. It consists of many two-dimensional pieces,” says Jarillo-Herrero, a fellow in the Quantum Materials program and a professor at the Massachusetts Institute of Technology in the U.S.
“Graphite is the material in a pencil, and when you write, it is almost like taking the deck, and spreading out the cards. A layer of graphene is like a single card, and at just one atom thick, it is the thinnest material.”
“Graphene comes from graphite, which is three-dimensional. But it is like a deck of cards.”
— Pablo Jarillo-Herrero
Graphite is a form of carbon, and before 2018, superconductivity had never been observed in carbon. But by aligning two graphene layers at a 1.1-degree angle, Jarillo-Herrero achieved it. It’s called magic angle graphene.
“Graphene is not a magnet, superconductor or insulator. But with different twist angles, we can make it have these properties,” says Jarillo-Herrero.
“Using graphene, and a material called hexagonal boron nitride, we have realized most quantum phases of matter. This is unique. No other material can realize all of these. Usually, you have to change the chemistry, or the material. But by twisting graphene, we can do it all in one.”
Research into two-dimensional twisted materials is still in its infancy. Jarillo-Herrero compares it to transistors in the 1940s. There is great potential, but we have only scratched its surface.“There are many challenges. We want to fabricate devices with different twist angles, and always hit the same angle. These systems are sensitive to tiny variations. An angle of 1.13 or 1.14 degrees is not the same, and we don’t have the technology to always make identical devices,” Jarillo-Herrero says.
Currently, these devices are made by graduate students, who Jarillo-Herrero compares to medieval monks who labour meticulously at their craft. He envisions a machine that automates and standardizes the fabrication process. He compares this to a Gutenberg press for quantum technologies. And just as the invention of an automated printing press ushered in an era of intellectual flourishing by greatly increasing the dissemination of the written word, Jarillo-Herrero views automation as critical to a fuller understanding of the potential of two-dimensional materials.
“A quantum press would automate stacking, and allow you to make materials in any combination, and at any angle. This would allow you to systematically test electronic, optical and mechanical properties. It takes my students several weeks to make each device, so it will take decades to explore this area. A quantum press would allow us to do this with the press of a button.”
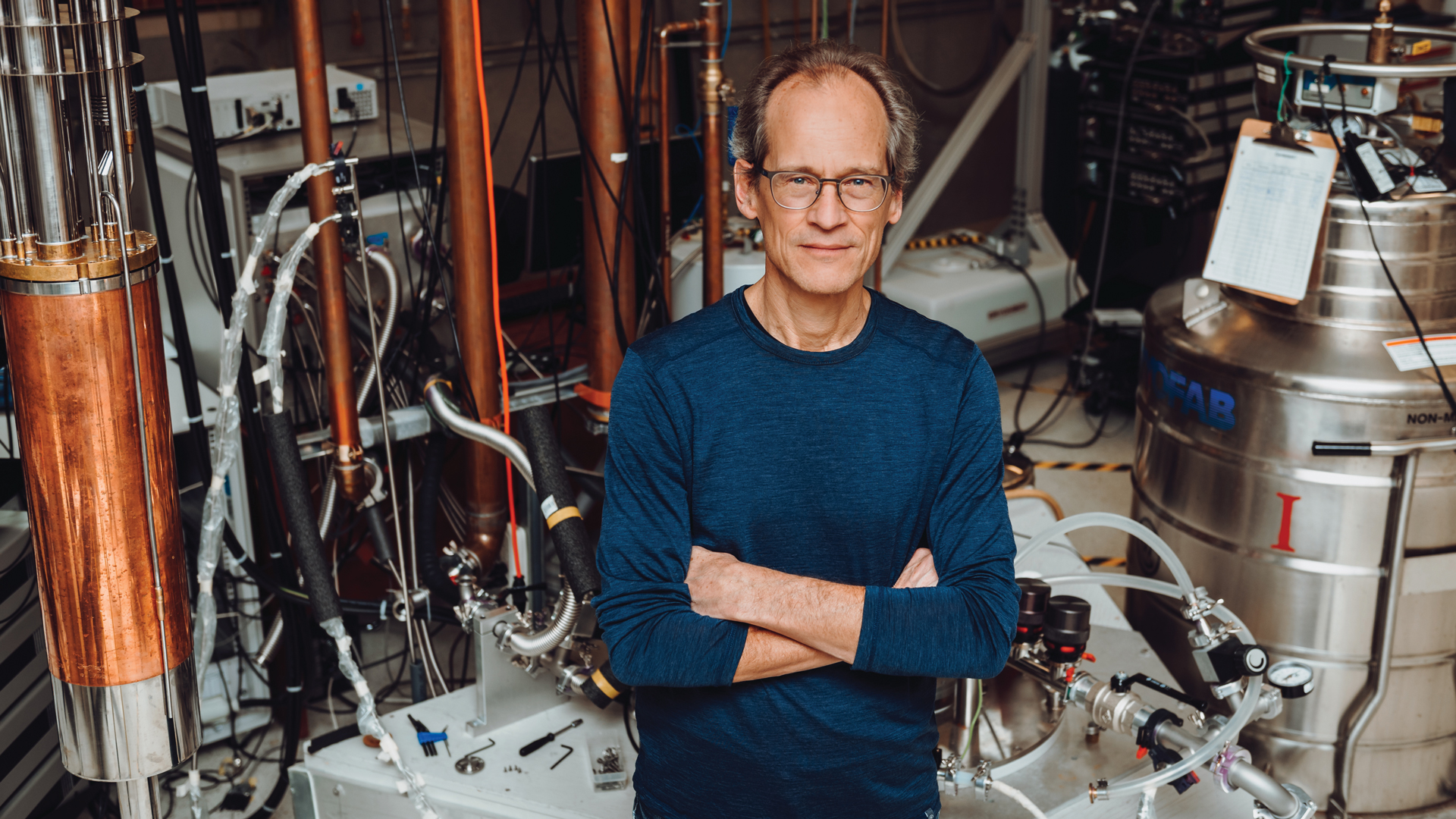
LOUIS TAILLEFER
In our existing electrical grid, a significant amount of electricity is lost during the transmission process because of the electrical resistance of the materials used in transmission wires. In practice, that means we generate a lot of electricity we don’t actually use.
Enter superconductors. A superconductor is a material that is able to conduct electricity with zero resistance. They could help solve this waste problem by eliminating the resistance, but there’s a problem in putting them to use. Superconductors only exhibit property below a critical temperature threshold, and for all known superconducting materials, that temperature is downright frigid.
Superconductivity was first observed all the way back in 1911, when Dutch physicist Heike Kamerlingh Onnes observed that mercury had no electrical resistance at the temperature of -269° Celsius. But that is roughly the same temperature as deep space, and led only to a few specialized applications, such as in magnets in MRI machines.
But in the 1980s, researchers identified a promising new type of superconducting material: copper oxides, also known as cuprates. These exhibited superconductivity at much higher temperatures — a still-very-brisk temperature of -140° C.
But it is possible to reach these temperatures with widely available coolants like liquid air, and this has enabled new applications. Cuprates are helping enable a clean energy revolution by making it possible to build wind turbines at sea.
“Nobody likes turbines in their backyard, and many are being built at sea. There is a lot of wind, and it’s out of the way, but also more delicate,” says Louis Taillefer, co-director of the Quantum Materials program and a professor at Université de Sherbrooke in Québec, Canada.
A superconductor that functions without complicated cooling equipment wouldn’t only transform power grids, it would enable applica-tions we haven’t even imagined. But to achieve this, we need a better understanding of cuprates’ quantum properties.
“You want to minimize a turbine’s weight. A superconductor can do this, while maintaining efficiency. It can reduce weight by a factor of five, but needs to be cooled with liquid nitrogen.”
The goal is to move beyond niche applications. A superconductor that functions without complicated cooling equipment wouldn’t only transform power grids, it would enable applications we haven’t even imagined. But to achieve this, we need a better understanding of cuprates’ quantum properties.
Cuprates are what is known as a strange metal — a type of material that defies the conventions of electrical conductivity. “Strange metals behave in baffling ways. They do not conform to our standard theory of electrons,” says Taillefer.
“There are many collisions between electrons, which seem to reach a maximum. When we try to measure the time between collisions, we find it given by Planck’s constant.”
Planck’s constant is fundamental to quantum mechanics. It was introduced in 1900 by the Nobel Prize-winning physicist Max Planck, who is considered the father of quantum theory. Planck theorized that light was constituted of individual particles that he called quanta of light. Today, physicists call these particles photons, and Planck’s constant is a calculation of the relationship between a photon’s energy and its frequency.
“The fact that Planck’s constant emerges as the shortest time electrons can have for collisions is leading us toward a new fundamental law of physics that we don’t yet understand. Strange metals behave in new ways. And we don’t know whether this could be harnessed for technology,” says Taillefer.
Superconductors have the potential for diverse applications because they behave as macroscopic quantum objects, meaning they have a single coherent quantum wave function.
“Think of it like a laser. What is the difference between a light bulb and a laser?” Taillefer asks.
“Both emit light, but a bulb does this in an incoherent way. Each photon is separate, and there are many different wavelengths and phases. But in a laser, every photon is in the same state — same frequency, same phase. We call this coherence. Superconductors are the electrical equivalent. All electrons are in a coherent quantum state. This is what makes them so powerful.”
Applications like power grids are obvious, but room temperature superconductors would open the door to entirely novel applications.
“When lasers were invented, nobody knew what to do with them. Now, they’re everywhere,” says Taillefer.
“This would happen with superconductors. When you need a specialized lab, few people can play with them. But once any kid can use their imagination with a superconducting object, the possibilities will multiply. It would be a technological revolution.”